Abstract
This paper is a synopsis of the general origins, causes, processes and structures of the Bishop Tuff eruption. The methodology of this paper will be to synthesize the results of previous studies of Long Valley volcanic systems to give a broad but concise overview of the Bishop Tuff eruption. This review has led to several conclusions based on both personal observations and on concurrence among the results of the studies cited, principally that the Bishop Tuff eruption was found to be a continuous, relatively sudden, explosive event that occurred about 760,000 years ago and that it originated from a singular horizontally and vertically zoned near-surface magma chamber developed via periodic basaltic heat injections over the course of several hundred thousand years; after the Bishop Tuff eruption, extensive volcanic activity, on a lesser scale, continued in and around Long Valley even as the magma chamber cooled and further crystallized. These results indicate that an eruption on a similar scale to Bishop Tuff is unlikely to be imminent, but that smaller-scale eruptions are a distinct possibility and that properly thorough risk-assessment studies must be undertaken to mitigate the volcanic hazard to the people of Long Valley Caldera and beyond.
Introduction
The Bishop Tuff/Long Valley eruption that occurred approximately 760,000 years ago was one of the largest volcanic events of the Quaternary era [Hildreth and Wilson, 2002]. In the span of six days, roughly 600 km3 of mostly rhyolitic material was emptied from the volcano’s magma chamber, creating a layer of welded material known as the Bishop Tuff that now covers an area of 2,200 km2. The ensuing collapse of the magma chamber’s roof led to the creation of a highly elliptical caldera 32 by 16 km wide and 2-3 km deep [Holahan et al., 2008]. After the cataclysm, the area has remained volcanically active, and is of considerable interest to geologists, as both a tool for learning about supervolcanic eruptions and as a present-day geological hazard that could pose a threat to human life.
Observations
Much of the information concerning the Long Valley supervolcanic eruption has originated from studies of its ignimbrite deposits, known as the Bishop Tuff. Comprising welded rhyolitic ash flows (and some ash fall), the Bishop Tuff covers an area of approximately 2,200 km2, mostly to the southeast of the caldera [Holahan et al., 2008]. Reaching a depth of up to 200 m, part of the Bishop Tuff forms one of Long Valley’s most recognizable landmarks-the Tablelands [Bailey, 1976]. Relatively exposed and eroded by the Owens River, the Tablelands offer a complete cross-section of deposited material.
Counter intuitively, the Bishop Tuff is not visible inside the caldera, but core sampling by the LVIEW (Long Valley Exploration Well) has indicated that the Bishop Tuff is up to 1,500 m thick inside the caldera, but has been buried by lake sediments and post-caldera volcanic fill [Shearer et al., 1995]. Indeed, up to 2/3 of the emitted material is thought to rest in the interior of the caldera.
The caldera itself is another notable topic of study. Measuring 32 by 16 km wide, the caldera is highly elliptical and remains to this day moderately topographically well defined, especially on its eastern edge. Bounded by Glass Mountain to the east and Mammoth Mountain to the west, the caldera is relatively flat, save for a series of raised post-eruption volcanic deposits near the center of the depression [Bailey, 1976].
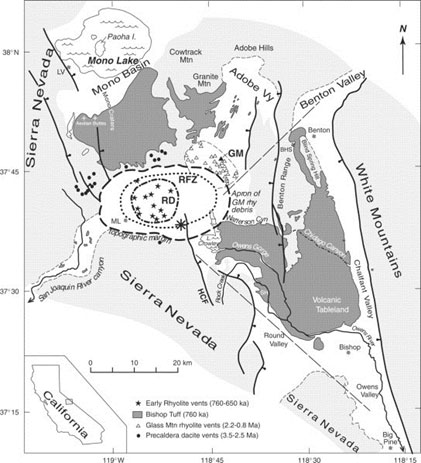
Figure 1: A Map of the Long Valley/Mono Lake Region with Dated Ignimbrite Deposits. [Holahan et al., 2008].
To the north of the Long Valley lies a chain of igneous extrusions stretching from Mammoth Mountain to Mono Lake known as the Mono-Inyo Craters. Relatively young in geologic terms (˜40,000 years), the Mono-Inyo system is composed of a medley of volcanic landforms, including phreatic craters, lava domes, and rhyolitic ash fall deposits [Bailey, 1976].
In addition to the Mono-Inyo Craters, the area in and around Long Valley remains tectonically and geothermally active, and volcanically sensitive. Hot springs and fumaroles remain relatively common features on the caldera floor, as do the remains of previous earthquake faults. Recently, starting in the early 1980s, there have been significant renewed periods of seismic and hydrothermal activity, with 4 quakes of approximately magnitude 6 occurring along the southern edge of the caldera ["Long Valley Caldera," 2012]. Several periods of crustal upwarping have also been observed on the caldera floor. On the flanks of Mammoth Mountain, CO2 emissions have been responsible for the deaths of several outdoorsmen.
Interpretations
Geologic Setting of Long Valley Caldera, Local and Regional
To preface discussion: the geologic conditions required for supervolcanoes to develop are not well understood, so some of what is said veers toward the speculative.
Long Valley is situated on the far western end (in the Owens Valley) of the Basin and Range Province, a large geologic system that envelops much of the western United States and is the predominant force in local geology [Bursik and Sieh, 1989]. The Basin and Range Province is characterized by large-scale tensional crustal stretching which results in extensive thinning of the lithosphere. A series of north-south oriented normal faults is a symptom of this stretching, as is horst and graben topography. Although no truly definitive consensus has emerged concerning the Basin and Range Province’s role in promoting supervolcanic conditions, it is widely speculated that the region’s thin continental crust provides an easier pathway for magma to rise near the surface. Additionally, Long Valley is located in a pull-apart basin, an area in which the intersection of two faults results in the subsidence of a rhomboidal area of land, and as such is possibly at higher risk of developing supervolcanoes [Bursik and Sieh, 1989].
Volcanic activity in the Long Valley Caldera area dates back (using K-Ar dating) to around 3.2 mya, and initially emitted mostly trachybasaltic-trachyandesitic material [Bailey, 1976]. Glass Mountain, the region’s oldest surviving volcano, formed about 2.2-.79 mya. During the latter stage of the Glass Mountain eruptions, from 1.1-.76 mya, the composition of the material extruded changed primarily to rhyolite.
This change in silica content is thought to correspond to the expansion of crystal mush and the injection of greater quantities of heat. Prior to the growth of the crystal mush, small rhyolitic "melt lenses" were isolated from the mush and varied somewhat in composition [Hildreth and Wilson, 2007). After 1.1 mya, the mush expanded, enveloped the melt lenses, and incrementally forced the creation of a larger, more homogenous chamber.
The Three Factors of Volcanic Eruptive Impact
In order to properly frame discussion of the nature of the Long Valley eruption, and specifically the properties of its magma chamber, the three underlying factors that caused the eruption to be so devastating must be examined. Colloquially known as "the three Vs," viscosity, volume, and volatile content are regarded as being the primary properties of magma bodies that influence the characteristics of an eruption.
The Three Factors of Volcanic Eruptive Impact
Viscosity
Viscosity is the measure of the relative "stickiness," or resistance to flow, of a liquid. Along with volatile content, viscosity influences the explosiveness of an eruption; the higher the viscosity, the greater the explosiveness, as a more viscous magma will better withstand pressure and thus be more energetic when ruptured. The primary factors in determining magmatic viscosity are interrelated: silica content and temperature ["Why Are Some Eruptions Gentle and Others Violent?"]. Increased silica (molecular formula: SiO2) content, which usually ranges from approximately 48-77%, results in more viscous magma. Mafic magmas (which have low silica content) are orders of magnitude less viscous than felsic magmas (which have high silica content). Simultaneously, temperature directly and indirectly influences the viscosity of magma; higher temperatures result both in re-melting precipitated crystals, thus lowering the silica content, and reducing the internal friction of the magma. Both effects correlate to lower viscosity. Conversely, lower temperatures result in a higher proportion of crystal precipitates and thus a higher silica content and more internal friction of the magma, resulting in higher viscosity. This process of changing melt composition through temperature is known as fractional crystallization.
Most of the material emitted during the Bishop Tuff eruption was highly rhyolitic. In the initial stage of the eruption, silica content hovered around 75% [Bailey, 1976]. Magma, especially toward the roof of the chamber, was relatively cool, with temperatures ranging from around 714°C to 816°C [Hildreth and Wilson, 2007]. This combination of low temperature and high silica content created conditions ripe for extremely viscous magma, which led to a highly explosive eruption.
Volume
The volume of pyroclastic material emitted is, intuitively, primarily responsible for determining the size (and to a lesser extent, the explosiveness) of an eruptive event. The quantity of material extruded from a volcanic event can vary tremendously from eruption to eruption; the volumes of lava flows from small shield volcanoes are often measured in terms of cubic meters, while supervolcanic events such as Yellowstone or Long Valley are typically measured in cubic kilometers.
he Bishop Tuff paroxysm was one of the largest expulsions of pyroclastic material known. The Mt. St. Helens eruption, the largest eruption on the contiguous lower 48 states in almost 100 years, produced approximately 1.2 km3 of material. Bishop Tuff, by comparison, extruded 600 km3 of tephra, the third largest release of volcanic material in the Quaternary United States ["Mount St. Helens," Bailey, 1976].
Volatile content
Volatile content, the percentage of dissolved gases in magma, is another factor in determining the explosiveness of an eruption. Dissolved gases such as CO2 and H20 come out of solution when the pressure in the magma drops below a certain point in a process known as "exsolution [Gonnermann and Manga, 2003]." During and after exsolution, gasses expand massively in volume, often violently quickly. Such rapid expansion results in magma being fragmented, often in the form of ash. Hence, the greater the amount of dissolved gas in magma, the more explosive the eruption.
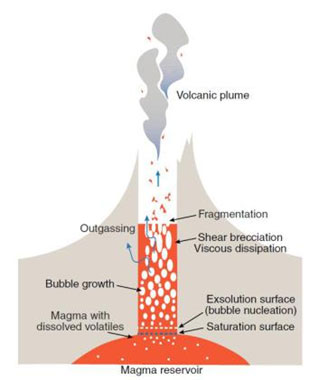
Figure 2: Exsolution of Volatiles [Gonnermann and Manga, 2003]
Especially near the roof of the magma chamber, the Bishop Tuff magma body was extremely gaseous. The high gas content of the magma most contributed heavily to the explosive power of the eruption [Hildreth and Wilson, 2007].
Bishop Tuff, Magma Bodies, and Rim Unzipping
The next several sections will deal with the conditions inside the Bishop Tuff magma body and how those conditions influenced the Bishop Tuff deposit. The topic of interest will be examining how the stratigraphy and composition of the Bishop Tuff can be interpreted in terms of magma body composition and the sequence of the eruption.
Bishop Tuff
The Bishop Tuff, as previously mentioned, is a rhyolitic ignimbrite that is relatively homogenous and unsorted, but is composed of four separate cooling units [Hildreth and Wilson, 2003]. These units are thought to have been expelled at different vents drawing from different latitudes and longitudes of the magma body. Consequently, their composition and temperature slightly varied, as did the degree of inter- and intra-unit welding. To elaborate, both the temperature of ejected material and the time intervals between ejections allowed for different degrees of welding. However, although there is evidence for numerous, separate ash flow deposits, the Bishop Tuff indicates that the eruption was relatively continuous for a period of six days, after which much of the volcanic activity subsided. A lack of rock weathering, silt deposits, and ash fall from other eruptions support this notion.
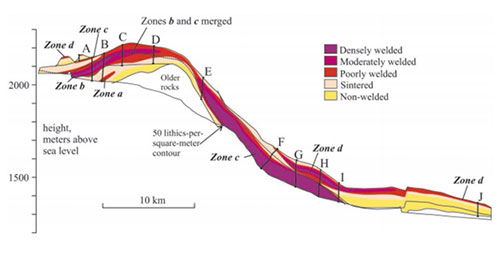
Logitudinal section down the line of the Owens Gorge projected on the true left side showing the overall welding structure of the ignimbrite. Density data from the 10 profiles (figs. 7-10) were combined with sparse point measurements and then matched to field observations at numerous sites along the gorge walls using the calibration between density, rock texture, and welding given in table 2. The dotted line is the 50 lithics/m2 contour derived from lithic numerical abundance data (fig. 5b), note how this contour serves to demarcate welding Zone b (best developed at sections A and B) and Zone c (best developed down–gorge from section E), with an overlap around sections C and D.
Figure 3: A Cross-Section of the Bishop Tuff Illustrating Differential Welding [Hildreth and Wilson, 2003].
Magma Body Conditions
The formation, structure, and internal mechanics of internal magma bodies is a hotly debated subject amongst geologists and as such the following information is a composite of interpretations from different scientists, and should not be viewed as a near certainty.
Most geologists accept the idea that the Bishop Tuff magma body was not uniform in temperature or composition and is instead thought to be both horizontally and vertically zoned by temperature, gas content, crystal content, and silica content [Hildreth and Wilson, 2003, Bailey, 1976]. These gradients are evident in the Bishop Tuff, as the most recently erupted parts of the deposit differ significantly in makeup to older deposits. Younger deposits have a higher crystal content (up to 25%, as compared to 5%), were exposed to higher temperatures (up to 818°C, from 714°C), and are less rhyolitic [Hildreth and Wilson, 2003]. This gradient suggests two premises for the eruption of the magma chamber: First, the expulsion of magma was sudden, rapid, continuous and brief, as the contents of the chamber clearly did not have time to re-equilibrate; re-equilibration would have produced a more homogenous deposit. Second, as the magma chamber emptied, the composition of the magma changed, which indicates the magma body was zoned. Phenocryst content (which ranged from 5-25%) and temperature (714-818°C) decreased in the higher levels of the chamber, and volatile and silica (74-77.7% silica) content rose toward the roof of the chamber. Thermal and gravitational forces are estimated to be the primary forces behind the creation of these gradients.
Roughly bounded, the magma body was 5-15 km deep [Hildreth and Wilson, 2007]. Surrounding the bottom half of the rhyolitic main core of liquidous magma was a large body of "plutonic crystal mush." This crystal mush was significantly less felsic than the rhyolitic interior, but contained an exceptionally high (˜50%) percentage of crystals. Due to its crystal content, the mush likely behaved more like a solid than a liquid. Underneath the bottom of the crystal mush, basalt has been hypothesized to have intruded sporadically, simultaneously injecting heat and new minerals into the chamber, contributing to the growth of both the crystal mush and rhyolitic melt. This crystalline mush is thought to have played a key role in the formation of the Bishop Tuff magma body (see "Geologic Setting of Long Valley Caldera, Local and Regional"). Basaltic heat injections have been linked to both horizontal zonation and initiation of eruptive sequences [Hildreth and Wilson, 2003]. Approximately 100 years prior to the Bishop Tuff eruption, an unknown volume of basalt was injected into the magma body, which conceivably was interrelated with the eruption [Wark et al., 2007]. Additionally, the series of powerful earthquakes centered in Long Valley during the early 1980s can possibly be viewed as a symptom of heat injection.
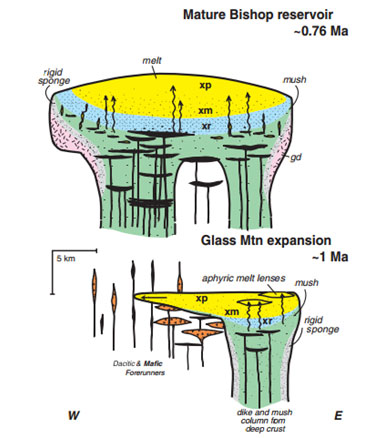
Figure 4: Progression of Approximate Dimensions of Magma Body from ˜1 to ˜.76 mya. [Hildreth and Wilson, 2007]
Eruption Sequence
The cataclysmic eruption is thought to have begun via opposite vents on the north and south flanks of the caldera. As material escaped, the roof began to sag, putting unsustainable sheer loads on the sides of the would-be caldera [Holahan et al., 2008]. Once the load became unsustainable, the roof of the chamber on each side of the vents "unzipped" and split open, following the approximate contours of the magma body. This unzipping continued until the opposite east-west poles of the caldera were jointly opened on either side, forming a series of roughly ovular fractures around the caldera (these fractures are known as "ring" fractures). Unable to support itself, the roof of the magma body subsided 2-3 km into the now empty of the space of the magma chamber, forming the Long Valley caldera. This sequence of venting, sagging, and unzipping is supported by scale analogous models with similar proportions to the Long Valley magma chamber. Such models suggest that the highly elliptical nature of the magma chamber was primarily responsible for the manner in which the chamber emptied, and that the formation of the ring fracture fault system was not influenced primarily by the location of local faults.
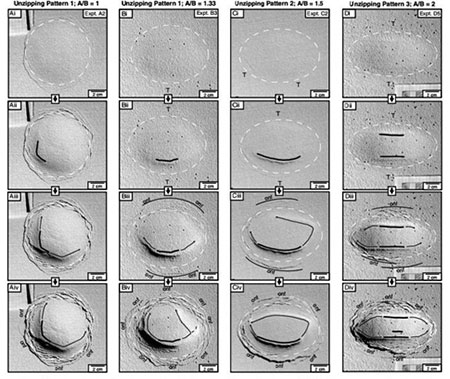
Time-lapse photos of experimental caldera collapses. These represent the main unzipping patterns observed, but note that A/B = 1.33 produced patterns 1 or 2, while A/B = 1.5 produced patterns 2 or 3. See Appendicies for full data set. AI-DI: prefailure sagging and surficial tension craks (T). Dashed white line is approximate chamber outline at depth. AII-DII: Localization of inner reverse fault(s) (trace indicated by thick black line). AIII-DIII: Lateral propagation of reverse fault trace(s) tracked by thin black line and arrows. Also see onset of outer normal faulting (onf-medium black lines). AIv-DIv: Completion of ring fault propagation.
Figure 5: A Series of Time Lapse Photos Detailing the Unzipping Process [Holahan et al., 2008].
Long Valley Post-Bishop Tuff
The Long Valley Caldera remained volcanically active post-Bishop Tuff and has maintained that level of activity to the present day. Almost directly after the Bishop Tuff eruption, smaller scale eruptions of rhyolitic (˜75% silica), crystal-poor "tuff, domes, and flows" occurred within the caldera for approximately 100,000 years [Bailey, 1976]. As with later eruptions, these early volcanic complexes generally follow north-south trending fault lines. Simultaneously, the magma body underneath these renewed centers of volcanic activity began to expand, causing the center of the caldera to rebound upwards until around 630,000 years ago, creating what is known as a "resurgent dome ["Long Valley Caldera," 2012]." Post resurgence, a series of lava domes and viscous lava flows composed of hornblende-biotite rhyolites erupted from 500,000-90,000 years ago [Bailey, 1976]. These flows have been causally christened "moat rhyolites." On the western edge of the caldera, Mammoth Mountain formed around 100,000-9,000 years ago from a series of rhyodacite (silica content 74-66%) flows and lava domes. Stretching north-south along a line of faults, the Mono-Inyo craters are the youngest and most active volcanic system in the Long Valley area. Mostly rhyolitic in content, Mono-Inyo have been described as a possible symptom of the early-stage refilling of the magma chamber [Bursik and Sieh, 1989].
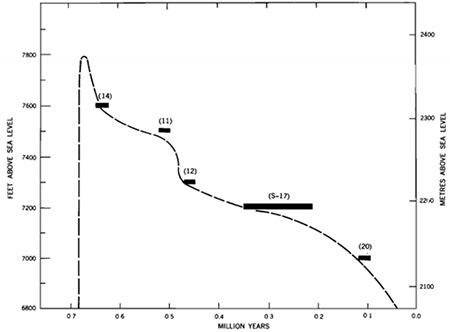
Elevations and ages of Pleistocene Long Valey Lake levels. Dashed line shows general rise and fall of lake level with time, based on age of associated volcanic unit (solid bar). Numbers in parentheses are locality numbers of dated samples (Table 1 and Figure 3). Length of bar is analytical error of age determination.
Figure 6: Graph of Pleistocene Long Valley Lake Level vs. Time [Bailey, 1976]
The present-day caldera is much more shallow and broad than the caldera that formed in the aftermath of the eruption. The alluvial (there was a large Pleistocene lake present in the caldera), volcanic, and erosional deposits that followed in the aftermath of the caldera’s collapse buried the Bishop Tuff in thousands of meters of sediment. Below the caldera, the magma chamber is thought to have partially cooled and crystallized due to heat loss [Bailey, 1976].
Conclusions
This paper is a synopsis of existing literature on the Bishop Tuff eruption and, as such, does not lend itself to strong original conclusions. However, the paper does synthesize studies from a number of sometimes disparate disciplines and provides a broad but concise summary of the research into the origins, causes, processes and structures of the Bishop Tuff eruption. The primary conclusion is that the Bishop Tuff eruption was found to be a continuous, relatively sudden, explosive event that occurred about 760,000 years ago and that it originated from a singular horizontally and vertically zoned near-surface magma chamber developed via periodic basaltic heat injections over the course of several hundred thousand years. Second, after the Bishop Tuff eruption, extensive volcanic activity, on a lesser scale, continued in and around Long Valley even as the magma chamber cooled and further crystallized.
Although this paper is most certainly not a detailed hazard analysis of the Long Valley area, some conclusions can still be drawn about the likelihood of an impending future supervolcanic eruption, namely that an eruption of the magnitude of the Bishop Tuff is unlikely to be imminent, but that smaller-scale eruptions are highly probable in the near future. Therefore, although an eruption on the scale of Bishop Tuff would be more devastating, it would be prudent to put significant emphasis on thorough risk-assessment studies that investigate the mitigation of the immediate potential volcanic hazard of smaller eruptions, such as those from the Mono Inyo chain, to the inhabitants of Long Valley Caldera and beyond.
References
Bailey, Roy A., Brent Dalrymple and Martin Lanphere. "Volcanism, Structure, and Geochronology of Long Valley Caldera, Mono County, California." Journal of Geophysical Research 81.5 (1976):725-744
Bursik, Marcus, and Kerry Sieh, "Range Front Faulting and Volcanism in the Mono Basin, Eastern California." Journal of Geophysical Research 94.B11 (1989):15,587-15,609
H. M. Gonnermann, M. Manga, "Explosive volcanism may not be an inevitable consequence of magma fragmentation." Nature 426 (2003), 432-435
Hildreth, Wes, and Colin J.N. Wilson. "Compositional Zoning of the Bishop Tuff." Journal Of Petrology 48.5 (2007):951-999
Holahan, Eoghan P., Valentin R. Troll, Benjamin van Wyk de Vries, John J. Walsh and Thomas R. Walter. "Unzipping Long Valley: An explanation for vent migration patterns during an elliptical ring fracture eruption." Geology 36.4 (2008):323-326.
"Mount St. Helens." USGS. November 12, 2012. Web. June 18, 2014.
Shearer, C.K, J.C. Eichelberger, J.J. Papike, M.J. Keskinen, P.W. Layer, and V.S. McConnell. "Rhyolite intrusions in the intracaldera Bishop Tuff, Long Valley Caldera, California." Journal of Volcanology and Geothermal Research 67 ( 1995) 41-60.
"Long Valley Caldera." USGS, May 5, 2012. Web. June 18, 2014.
Wark, D.A., W. Hildreth, F.S. Spear, D.J. Cherniak and E.B. Watson. "Pre-eruption recharge of the Bishop magma system." Geology 35.3 (2007):235-238.
"Why Are Some Eruptions Gentle and Others Violent?" Volcano World, Oregon State Department of Geosciences. Web. June 18, 2014
Wilson, Colin J.N., and Hildreth, Wes. "Assembling an Ignimbrite: Mechanical and Thermal Building Blocks in the Bishop Tuff, California." The Journal of Geology 111(2003):653-670.