Many places exist on planet Earth that are considered harsh, extreme, or otherwise uninhabitable. Scientific advances in the past 50 years have shed new light on these environments, giving us a better understanding of the conditions in extreme places. Scientists have also discovered various life forms with a diverse range of adaptations that flourish in these places. Thriving in hot, cold, high pressure, saline, alkaline, acidic, and anaerobic places, these organisms broaden the concept of habitable environments to be more inclusive than previously thought.
Introduction
The tops of mountains; the bottoms of oceans; permanently frozen soil; boiling, magma-heated waters. All over the world, places exist that are uninhabitable by human standards, and not just for humans. Some water is too salty even for fish, and even polar bears get cold at some point. There are some parts of the planet that are just too (hot, cold, acidic, alkaline, salty, deep) extreme for life. Right?
Scientists have been trying to define the limits of life for a while now, but we still don’t know them for sure. The assumption is that there are certain thresholds, beyond which life–sustaining processes will cease to happen. Above a certain temperature the molecules that compose most organisms will fall apart. Below a certain temperature most chemical reactions slow to the point of virtually stopping. At one time it was proposed that life could only exist in temperatures that generally stayed between 0°C and 50°C [Postgate, 1994]. Since then scientists have discovered organisms that thrived well above 50°C, well below 0°C, and in all sorts of unlikely places (See Table 1). Obviously the hypothesis was incorrect, but where did it go wrong?
For a long time, humans could only study what they could see with the naked eye. In biology this meant macroscopic organisms such as plants and animals. It wasn’t until the 17th century that microscopes could magnify subjects enough to view them at the cellular level [Egerton, 2006]. Anton Van Leeuwenhoek, known to some as the Father of Microbiology, first observed microorganisms living in lake water in 1694. Even once we could see into this new microscopic world, scientists could only study samples from places they could access (i.e. not deep sea trenches). In general, this meant that the kinds of life we knew about lived in about the same temperature range and at about 1 atmosphere of pressure.
It is not unreasonable to assume that this kind, our kind, of life does have certain limits. Temperate species have adapted to live in conditions characteristic to Earth’s surface, and in a different environment their machinery might not function correctly [Postgate, 1994]. However, just because an environment is unsuitable for certain types of life, doesn’t necessarily mean it’s unsuitable for all life. The organisms that live in these extreme (formerly considered inhospitable) places are especially suited to them, and are appropriately called extremophiles.
The term extremophile encompasses a wide variety of organisms from all three domains of life (eukarya, bacteria, and archaea) [Rainey and Oren, 2006]. Extremophiles contain DNA and depend on water to function, just like all life on Earth; however, they have unique compounds, habits, and metabolic processes that allow them to live in places the rest of us cannot. There are seven main categories of extremophiles: thermophiles, psychrophiles, barophiles, halophiles, alkaliphiles, acidophiles, and anaerobes. In practice, these categories aren’t mutually exclusive. Often times, multiple extreme conditions will occur together like an acidic, superheated sulfur vent, or an extremely cold, high pressure ocean trench. For the purposes of this paper, however, it makes sense to separate them in order to gain a general understanding of these diverse organisms.
Extremophiles are defined by the environments in which they thrive. To better understand the extremophiles it helps to have knowledge about their habitats. In this paper I will describe some of the environments extremophiles inhabit, and explain how these extreme places exist on a planet that seems otherwise temperate. I will also explain some of the special talents extremophiles use to cope with these environments.
Table 1. Extremophile record holders as of 2006. Figure adapted from Rainey and Oren [2006](modified reproduction).
Hot Places
Most of the Earth’s surface doesn’t get much hotter than 45°C [Postgate, 1994]. The main exception to this rule is hydrothermal vents, which usually form in volcanic areas. Groundwater in or near a magma chamber becomes superheated and works its way up through openings in the crust [USGS website, 2013]. It releases as fumaroles, geysers, or hot springs, depending on the specific conditions of the discharge point (See Figure 1). Fumaroles are steam vents where the water has vaporized before it reaches the surface. Geysers and hot springs both occur when the hot groundwater discharges into a surface water source such as a river or lake. A geyser is a tall column of steam and hot water that shoots up into the air when enough pressure builds up underground. Hot springs occur when the heated groundwater mixes with cooler surface water in a less explosive manner. The temperatures vary from one spring to another as illustrated by Hot Creek in the Long Valley Caldera in California. At spots such as the Hot Creek fish hatchery the cold surface water is only raised about 5 degrees by hydrothermal vents, but the bluest hottest pools can be upwards of 70°C, over 30 degrees warmer than the ambient surface water temperature.
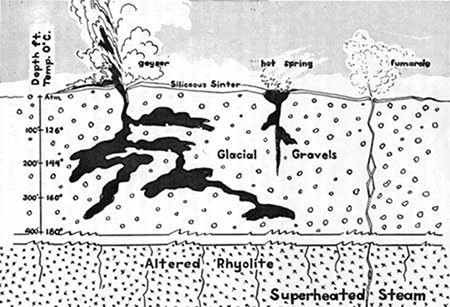
Figure 1. Different water paths that could lead to different hydrothermal features. "Cross Section of View of a Typical Geyser Basin. Depth and temperature data based on Fenner (1936)." Figure reproduced from Yellowstone Nature Notes [2006] (exact reproduction).
Organisms that live in environments hotter than 50°C are called thermophiles, heat lovers [Postgate, 1994]. 50°C is approximately the temperature at which proteins denature (lose their shape) in the cells of animals, plants, and other temperate organisms. It is also around this temperature that the waxy, fat component of the cell membrane begins to melt, and the cells are no longer able to regulate which substances enter and leave. Thermophiles don’t have these problems because they are made of different materials that can withstand the heat. They often contain chaperonins, proteins that are heat resistant and also protect other proteins that are not as heat resistant. In their cell membranes thermophiles have the fat–like substances ethers, with high melting points, in place of ethers, the fats in normal cells.
At about 70°C the DNA double helix splits down the middle, yet Thermus aquaticus can live in up to 80°C water, and other organisms have been found in even hotter settings [Postgate, 1994]. At hydrothermal vents deep in the ocean an organism called Pyrolobus fumarii functions best at 106°C, and can survive up to 113°C [Rainey and Oren, 2006]. No one has discovered exactly how their DNA can keep its shape at these temperatures, but one possible explanation might be that chaperonins stabilize not only the thermophile’s proteins but the DNA double helix as well [Postgate, 1994]. Thermophiles are also found in compost and trash heaps, refuse from industrial processes and mining, as well as the deep subsurface of the Earth [Rainey and Oren, 2006].
Cold Places
Below its surface, the vast majority of ocean water is cold [Margesin and Miteva, 2011]. In fact 90% of seawater never gets warmer than 5°C. In high mountains, thin air and high winds can keep the temperature cool enough that snow never melts. Permafrost (permanently frozen soil) can drop to -40°C, and glacial ice can range from -10°C down to -56°C. In short, the Earth contains many very cold places; places that are too cold for most organisms, but perfect homes for cold–loving psychrophiles. Psychrophiles thrive in environments that bring most life–sustaining processes to a halt [Postgate, 1994].
One famous psychrophile, the cause of "watermelon snow," is found in high mountains like the Alps or the Sierra Nevadas [Remias et al., 2005]. This pink snow is the result of photosynthetic algae that contain of red carotenoids. Chlamydomonas nivalis thrives in freezing water. It’s often seen in the summertime, in persistent snowfields that are a mixture of snow and melt–water.
As temperatures drop, chemical reactions slow, but psychrophiles use enzymes optimized for low temperatures to avoid the problem [Postgate, 1994]. When water, a primary component of most cells, freezes it expands into ice. Ice crystals easily rupture cells. Psychrophiles can live at temperatures well below freezing, however, because they are composed of substances like glycerin, that have much lower freezing points than water. When glycerin does freeze, it doesn’t expand into a crystal like water. It solidifies in place, similar to glass, keeping its shape and size. A frozen psychrophile remains dormant until the glycerin thaws and it can resume functioning. Psychrophiles are composed of substances that stay fluid in subfreezing temperatures. The downfall of these adaptations is that parts of them can melt at higher temperatures. Most psychrophiles die above 20°C.
High Pressure Places
In the atmosphere, pressure increases as elevation decreases. In water the effects of pressure are exaggerated by the weight of liquid, a phenomenon called hydrostatic pressure [Postgate, 1994]. In some ocean trenches pressure can be 1100 times greater than at sea level, strong enough to crush cars. Despite the crushing force, life exists nearly everywhere we’ve looked on the sea floor. From clams, crabs, and tubeworms clustered around hydrothermal vents, to microbes floating in near freezing water, nature has shown that high pressure is a non–issue. Barophiles, as these creatures are known, have also been found deep in the Earth’s surface [Impey, 2007].
Interestingly enough, many microscopic creatures from the seafloor can adjust to sea level pressure as long as the temperature is correct, and many sea–level microbes have been shown to thrive at 300 atmospheres of pressure [Postgate, 1994]. They might even persist at higher pressures, but it’s difficult and dangerous to create these conditions in a lab. Larger deep sea creatures have a variety of different adaptations that allow them to withstand the conditions, but on the microscopic level it doesn’t appear that anything special is going on. Unicellular organisms do not have organs and structures that get crushed the same way larger organisms do. Pressure doesn’t seem to affect microbes much at all.
Salty Places
In the western United States a hydrologic zone exists called the Great Basin [USGS website, 2013] (See Figure 2). Including parts of Utah, Nevada, and California, the Great Basin is surrounded by high lands on all sides like the Sierra Nevada Range and the Colorado Plateau. Water behavior within the Great Basin is peculiar, because it does not flow to the sea [Hart, 1996]. The water has no way of defying gravity to climb up over the mountains and spill into the Pacific Ocean or Gulf of Mexico. Instead it collects at low points in the landscape, forming terminal lakes like the Great Salt Lake in Utah, or Mono Lake in California. They are called terminal lakes because they do not have any outgoing streams. Since water can’t flow out of the Great Basin, the only outlet for water from terminal lakes is evaporation.
The Great Basin also happens to be in a rain shadow cast by the Sierra Nevadas [Hart, 1996]. Very little moisture makes it over the mountains to precipitate in the Basin directly. Instead, most of the water that feeds into the lakes is runoff from mountains when snow melts in the springtime. As the spring melt runs across rocks, dirt, sand, and soil it dissolves salts which it carries to the lakes. When water evaporates out of the lakes, solutes are precipitated out and left behind. Over time salts build up in the lakes to the point that they are inhospitable to many creatures. Actual salt concentrations vary by lake. For example, Mono Lake is three times saltier than sea water, while Great Salt Lake is ten times saltier. The salinity is affected by how much water flows into the lake, the rate of evaporation, and the age of the lake, among other things. The water in these lakes is so salty, even fish that live in the ocean cannot survive in them.
Salt lakes were long considered "dead" lakes by society and the scientific community [Hart, 1996]. On further inspection this idea was found to be incorrect. Salt Lakes are actually some of the most productive ecosystems known. Creatures that live in them are called halophiles (salt lovers) [Postgate, 1994]. They thrive in brine that would suck other organisms dry. Most organisms cannot handle the high osmotic pressure of hypersaline solutions, which tend to draw water out of the cells. Halophiles have developed different ways to counteract this, often by creating ion–rich solutions within their cells to balance out internal and external osmotic pressure. Halophiles excel at selectively accepting high ratios of potassium to sodium from their environment, despite the much higher frequency of sodium in nature.
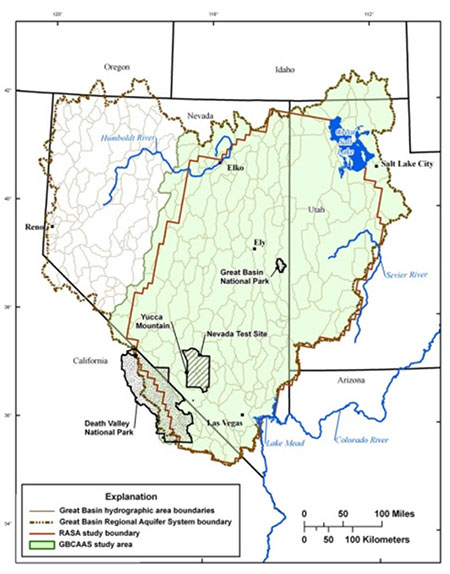
Figure 2. Boundaries of the Great Basin. Figure reproduced from U.S. Geological Survey website [2013](exact reproduction).
Some organisms such as red Halobacterium are obligate halophiles [Postgate, 1994]. They actually require high saline environments to survive, and will die in freshwater. Other microbes like Halomonas elongata are more flexible. H. elongata thrives in solutions anywhere from 0.3% (nearly freshwater) to 33% (the point of saturation) [Rainey and Oren, 2006]. Dunalliela is halophilic green algae that forms the base of many food chains in hypersaline environments [Impey, 2007]. In addition to salt lakes, halophiles also thrive in coastal lagoons, mineral salt deposits, and saline soils [Rainey and Oren, 2006].
Alkaline Places
Mono Lake, in addition to high salt concentrations, contains large amounts of carbonate ions, making it alkaline as well as hypersaline [Hill, 2006]. In fact, at pH 10-11.5, Mono Lake is eighty times more alkaline than ocean water. By the same process that supplies Mono Lake with salts, spring mountain runoff carries sodium carbonate compounds from the rocks. Carbonate levels increase as water evaporates and dissolved solutes build up.
Alkaline environments offer their own unique set of survival challenges. In particular, alkali tends to break down the fat in cell membranes and create soap [Postgate, 1994]. Creatures not designed for such high pH environments will slowly dissolve. Alkalophiles have cell membranes made of alkali–resistant fats, or they might have a fat replacement that does not react with alkali. Cells also require very strict internal pH levels and are very selective in accepting protons and hydroxyl ions through their membranes. Normal cells require pH 7.7, just slightly alkaline. Alkalophiles do not have an internal pH as alkaline as their surroundings. They excel at sorting ions to keep a more neutral internal environment; however, they still make some concession to their surroundings by keeping internal pH at about 9. Their enzymes and proteins have been shown to function best in this alkaline environment.
Acidic Places
In the 1860s Iron Mountain Mine was opened in Shasta County, California to harvest a large iron ore deposit [Superfund site overview, 2013]. During its lifetime Iron Mountain was mined intermittently for silver, copper, gold, zinc, and pyrite. It was effectively abandoned in 1963, and all the waste and tailings were left out in the open [EPA, 2006]. Iron Mountain was hugely productive, rich with heavy metals valuable to many different industries. It is, in fact, a "massive sulfide deposit," volcanogenic in origin. Four hundred million years ago, underwater hydrothermal vents spewed sulfur substances from deeper layers of the Earth onto the sea floor. These substances settled, forming deposits that later became Iron Mountain.
Sulfur is a rather common element in Earth’s crust, and it is also a favorite food of Thiobacilli [Postgate, 1994]. Thiobacilli is a group of bacteria that can metabolize sulfur substances. These bacteria live in sulfur deposits and soils, but also tailings like those at Iron Mountain Mine. They feed primarily on the abundant pyrite (iron sulfide) and produce sulfuric acid as waste. Sulfuric acid is one of the "strong" acids, and Thiobacilli eventually produce an environment full of it. With a pH of 1, this environment is uninhabitable to most organisms besides other acidophiles.
Acidophiles, such as Thiobacilli, are specially designed to cope with low pH [Postgate, 1994]. They have the opposite challenge of alkalophiles, where they must keep out most hydrogen protons or they will become too acidic internally [Rainey and Oren, 2006]. Like alkalophiles they make a slight concession to the environment, in their case keeping an internal pH of 5, lower than the normal 7.7 [Postgate, 1994].
Iron Mountain Mine was an EPA superfund site referred to as a "worst case scenario" [EPA, 2006]. Sulfuric acid and heavy metals were leaching from tailings piles into the local water supply. After careful study, Thiobacilli, Ferroplasma acidarmanus and others were discovered to be the culprits, giving acidophiles a claim to infamy as the polluting microbes [Impey, 2007; EPA, 2006].
Places Without Oxygen
Many terrestrial and aquatic creatures today depend on oxygen to survive. Luckily for them, much of the Earth’s surface has oxygen gas easily accessible: the atmosphere we breathe is about 20% O2 gas [Create a new climate, EPA], and bodies of water carry dissolved oxygen as well [Postgate, 1994]. The oxygen breathers that live here are called aerobes. There are also parts of the planet that contain very little to no oxygen, and they are more ordinary that one might think. Anaerobic (oxygenless) environments commonly occur in places that are decaying, such as mud, dung, and compost heaps. These places tend to be nutrient–rich, encouraging vigorous aerobic microbe growth, until they use up the oxygen during respiration. This provides the perfect low/no oxygen, high–nutrient environment that anaerobic bacteria thrive in.
Anaerobic bacteria "breathe" or metabolize other substances besides oxygen. They have many different processes like fermentation and nitrate, carbonate, sulfate, iron, or mineral compound reduction [Postgate, 1994]. Facultative anaerobes are those that can survive in low or no oxygen environments, but also do well in oxygenated places. Obligate anaerobes are those that can only survive without oxygen. When oxygen is present, it quickly reacts with their cells, corroding and destroying them.
Today’s anaerobes are thought to be the descendants of ancient life forms that existed before oxygen became a major part of the atmosphere [Postgate, 1994]. After the evolution of the now dominant aerobic organisms, anaerobes persisted in small, oxygenless places up to present day.
Conclusion
As technology improves, life is discovered in more and more unlikely places every year [Impey, 2007]. The limits scientists once set on temperature, pressure, and other conditions for life have been broken again and again. Our knowledge of the diversity and adaptability of life continues to expand. If hydrothermal vents and salt lakes are any example, we will continue to discover new "extreme" places that life thrives. As people explore deeper, higher, and farther, the rules of what constitutes a "habitable" environment become more and more inclusive. Perhaps we will reach a point where the "extreme" environments of today are no longer extreme, as new conditions are encountered as we continue to explore the universe.
References
Create a new climate for action: Glossary of climate change terms. website
Egerton, F. N. (January 2006). A history of the ecological sciences part 19: Leeuwenhoek’s microscopic natural history. Bulletin of the Ecological Society of America, 88, 47-58. DOI
Environmental Protection Agency. (2006, March 7). Abandoned mine lands case study: Iron Mountain Mine, success through planning, partnerships, and perseverance. website
Hart, J. (1996). Storm over mono: The Mono Lake battle and the California water future. Berkeley and Los Angeles, California: University of California Press.
Hill, M. (2006). California natural history guides: Geology of the Sierra Nevada. Berkely and Los Angeles, California: University of California Press.
Impey, C. (2007). The living cosmos: Our search for life in the universe. New York: Random House.
Margesin, R., and Miteva, V. (2011). Diversity and ecology of psychrophilic microorganisms. Research in Microbiology, 162, 346-361. DOI
Postgate, J. (1994). The outer reaches of life. Cambridge, Great Britain: Cambridge University.
Rainey, F. A. Editor & Oren, A. Editor (Eds.). (2006). Methods in microbiology: Extremophiles (Vol. 35). Sandiego, CA: Elsevier.
Remias, D,. Lutz-Meindl, U., Lutz, C. (August 2005). Photosynthesis, pigments, and ultrastructure of the alpine snow alga Chlamydomonas nivalis [Abstract]. European Journal of Phycology, 40(3), 259-268.
(2013, June 8). Superfund site overview Iron Mountain Mine, pacific southwest, US EPA. website
U.S. Geologic Survey Website. (27 Feb. 2013) US Department of the Interior. retrieved from: website on (6 June, 2013).
(2006, March 6) Yellowstone Nature Notes. website